Cardiac Imaging using the Chromacity 1040
Application Note Summary
Multiphoton microscopy has been demonstrated as a versatile tool in the life sciences for many structural and functional applications both in-situ and in vivo, including electrophysiology [1], calcium signalling [2], blood flow [3] and immune cell trafficking in the heart in vivo [4]. Despite the analytic power of such devices, and their increased availability both as commercially and as a custom-built device, their uptake in biology labs remains relatively low in comparison to the sister technology of confocal microscopy. This is in part due to the size, cost, and perceived complexity of purchasing and maintaining the most crucial piece of equipment for two-photon microscopy; the laser.

While expensive and bulky Ti:Sapphire lasers remain the most commonly used laser for two-photon applications, there is an increasing need for low cost, compact, and commercially available turnkey solutions capable of delivering higher average power at wavelengths >1000nm, where the traditional tunable Ti:Sapphire system struggles to deliver. This is demonstrated by electrophysiological measurements in excitable tissues, particularly cardiac and neural tissue. This frequently involves recording membrane potentials and calcium transients via multiphoton processes. For this purpose, a broad range of voltage [5] and calcium-sensitive [6] fluorophores have been specifically developed.
However, these processes are in the second IR imaging window (1 - 1.3μm), a wavelength range where the power of Ti:Sapphire systems falls off dramatically (Figure 1). In this application note the use of a low cost commercially available turnkey laser system from Chromacity is demonstrated. It is capable of delivering high peak and average powers at the sample plane of a two-photon microscope.
Introduction
Two-photon imaging for in vivo applications has been shown to achieve high-resolution imaging and execute functional measurements within neuroscience [7]. Such functional measurements are also being performed in other excitable media such as cardiac muscle, which exhibits even greater absorption and scattering [8]. Despite the relatively high tissue penetration depths afforded by this technique there remain fundamental limits to the depth of imaging possible, which are a function of the detection characteristics of the microscope, the optical properties of the tissue being imaged, and more importantly the pulsed laser source. The increased requirement for deep-tissue in vivo imaging has also spurred the development of genetically encoded sensors for red-shifted imaging [9]. The signal to noise requirements for fluorescent electrophysiological recordings are much lower than that required to resolve a detailed structural image. Therefore, during purely electrophysiological measurements, the ability to deliver sufficient power to a confined region deep within certain tissue types becomes more important than resolving individual structural features. This is particularly true in electrically well-coupled tissue such as cardiac muscle, hence, the need for suitable optical powers being delivered to the sample plane.

Optical Setup
A schematic of the optical layout is shown in Figure 2. An ultrafast ytterbium fiber laser from Chromacity capable of delivering 100fs pulses at 80MHz repetition rate, was coupled to a Zeiss LSM510 laser scanning microscope system. Laser output power was manually controlled using a Chromacity power attenuation module. Beam divergence was compensated for using a divergence adjustable beam expander. The beam was focused into the LSM510 scan head. The use of a 90° flip-mount and mirror allowed the beam path to be switched rapidly between the Chromacity 1040 and a tunable Ti:Sapphire.
Isolated perfused heart preparation
Hearts from three animals were used in the study. The whole heart preparation was set up and imaged as described in [10]. Hearts were bolus loaded with 25μl of di-4-ANEPPS (2mM stock) over a 10 min period. Imaging of cardiac structures was performed using a 40X mag, 1.0 NA objective lens. Two bi-alkali photomultiplier tubes (PMT) were used to capture the dual emission from di-4-ANEPPS. PMT 1 collected emitted light between 510-560nm while PMT 2 collected the longer 590-650nm emission band. A series of x-y images were recorded using each laser to form an image stack (z-stack). Afterwards, rapid line scanning at 1kHz was used to provide sufficient bandwidth to record optical action potentials. A ratio of both PMT signals was then taken to reduce motion artifact and baseline drift. This process was repeated sequentially, in 50μm steps, at increasing transmural tissue depths from 50 to 250μm below the epicardial surface.
Results
Pulse characteristics were determined initially using autocorrelation techniques. The autocorrelation for the Ti:Sapphire yielded a pulse duration of 120fs. The design of the Chromacity 1040nm system features a movable diffraction grating, resulting in pre-chirping of the pulse to compensate for dispersion effects through the microscope optics and maximise the fluorescence signal at the sample plane. Once the beam was aligned with the microscope scan head the diffraction grating was adjusted to yield the maximal fluorescence from a test sample of 500μm fluorescent beads.
Laser beam profiling measurements demonstrated that the beam waist of the Ti:Sapphire (Figure 3A) was roughly double that of the Chromacity 1040 (Figure 3B). As a consequence, the Chromacity 1040 beam diverged more rapidly than the Ti:Sapphire along its beam path. The Chromacity 1040 optical path therefore included a divergence adjustable beam expander. This yielded two photon-excited fluorescent signals with intensity matching the Ti:Sapphire at 1040nm (PMT gain and average power at sample plane matched). This was confirmed from z-stack images taken through the left ventricular epicardial surface at 15mW average power (Figure 4 and 5).

Due to the length of each scan, z-stacks for each laser were taken at adjacent regions of tissue to avoid any disparity in signal due to dye bleaching. The average signal from a defined imaging region was almost identical at each z-stack image plane for both lasers when power and wavelength matched (Figure 5B).
Optical action potentials recorded from a series of transmural depths with both lasers using rapid line scanning are shown in Figure 6A. Average power at the sample plane was relatively low (25mW) close to the epicardial surface (50μm z-depth). Here both lasers performed equally well at 1040nm, producing action potentials of comparable amplitude.
From depths of 100-250μm, power was increased on the Ti:Sapphire to maximum, yielding 60mW at the sample plane. Figure 6B shows the drop in action potential amplitude with depth, to the extent that signal to noise was insufficient at 200μm below the surface to record a usable action potential. Increasing the power of the Chromacity 1040 to 75mW, 15mW above the Ti:Sapphire power threshold at 1040nm, allowed action potentials of sufficient signal to noise to be recorded as deep as 250μm (data not shown), without damaging the tissue sample.

Discussion
Many system builders still opt for bundling microscopes with a tunable, solid-state Ti:Sapphire laser which, while offering flexibility, is bulky, costly to purchase and requires regular maintenance. Recent advances in fiber lasers have led to a significant increase in the use of this technology as an efficient, low cost alternative to traditional solid-state lasers, albeit limited to single wavelengths. Low cost custom-built fiber lasers for two-photon applications have been demonstrated for as little as $13,000 [11], but remain within the realm of specialist users with the appropriate knowledge and skillset to construct them.
In the setup shown in this application note the Chromacity 1040nm system can deliver >400mW at the sample plane, whereas the Ti:Sapphire can only deliver 60mW. While 400mW is in excess of what biological tissue can tolerate, it is likely that increasing the power beyond 75mW at deeper transmural tissue planes would increase the range of imaging of the system even further than that reported while remaining within the tolerable energy limits for intact cardiac tissue.
The flexibility of this laser system is illustrated by the relative ease with which it was coupled into the existing microscope scan head, without any prior knowledge of the optics within. The footprint of the system is low, occupying almost 2.5x less optical table space than the Ti:Sapphire and requiring only a single panel-mounted control unit. In addition, the system is passively air-cooled, eliminating maintenance costs and further reducing the overall footprint of the two-photon microscope.
In this experimental setup, the Chromacity 1040nm system can deliver >400mW at the sample plane, whereas the Ti:Sapphire can only deliver 60mW.

Conclusion
Comparing the Chromacity 1040 to the commercially available solid-state Ti:Sapphire at 1040nm the Chromacity 1040 was able to perform equally well and in some cases better. The Chromacity 1040 was easily able to meet the high power demands of deep tissue optical electro-physiological imaging. Through the use of a commercially available high-power ytterbium fiber laser at 1040nm relative to existing Ti:Sapphire lasers, significant improvements in transmural, deep tissue imaging of cardiac tissue can be gained. The Chromacity 1040 laser platform offers ease of use and installation into a laser scanning microscope system and is suitable for new and existing microscopes alike. For users wishing to purchase an entirely new system the fiber laser option reduces space considerably and brings the total cost for a complete basic laser scanning two-photon system down to between £100,000 - £120,000, close to the price of a single Ti:Sapphire laser alone. Those with an existing microscope who require more power for deep tissue imaging at wavelengths >1000nm could also consider this as an option thanks to the compact size of the Chromacity 1040 system.

All procedures were approved by the Institutional Ethics Review Board and carried out in accordance with the UK Animals (Scientific Procedures) Act 1986.
With thanks to Dr. Allan Kelly and QuantIC at the University of Glasgow.
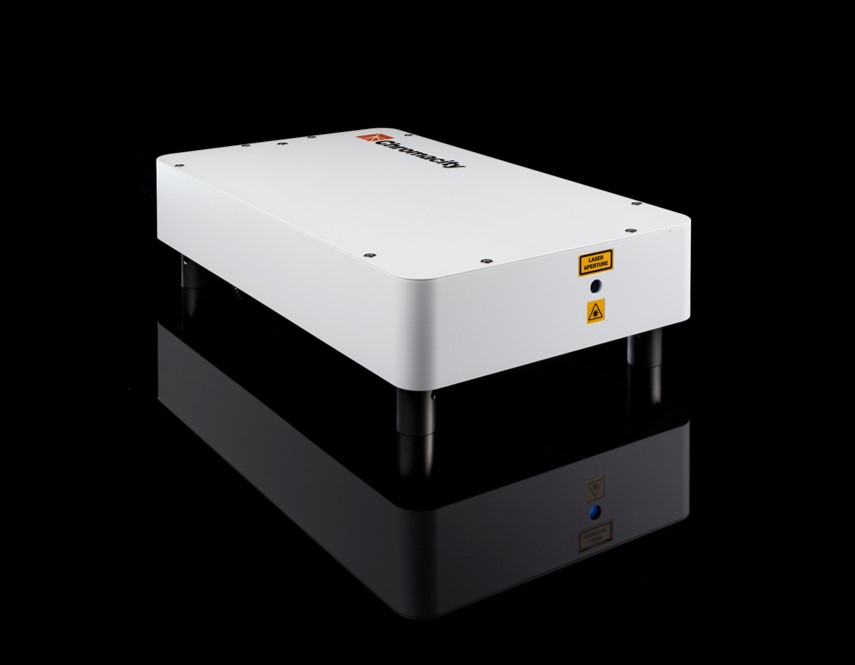
Chromacity 1040 Ultrafast Laser
- Compact, power-efficient ultrafast laser (<150 fs) with long-term output stability
- User-friendly setup with intuitive interface and space-saving laser housing
References
- J Biophotonics. 2015; 8:112-123. Ghouri IA, Kelly A, Burton FL, Smith GL, Kemi OJ. “2-photon excitation fluorescence microscopy enables deeper high resolution imaging of voltage and Ca2+ in intact mice, rat and rabbit hearts.”
- Microsc Microanal. 2008; 14:492–506. Scherschel J a, Rubart M. “Cardiovascular imaging using two-photon microscopy.”
- J Cereb Blood Flow Metab. 2012; 32:1277–1309. Shih AY, Driscoll JD, Drew PJ, Nishimura N, Schaffer CB, Kleinfeld D. “Two-photon microscopy as a tool to study blood flow and neurovascular coupling in the rodent brain.”
- J Clin Invest. 2012; 122:2499–2508. Li W, Nava RG, Bribriesco AC, Zinselmeyer BH, Spahn JH, Gelman AE, Krupnick AS, Miller MJ, Kreisel D. “Intravital 2-photon imaging of leukocyte trafficking in beating heart.”
- Proc Natl Acad Sci. 2012; 109:20443–20448. Yan P, Acker CD, Zhou W-L, Lee P, Bollensdorff C, Negrean A, Lotti J, Sacconi L, Antic SD, Kohl P, Mansvelder HD, Pavone FS, Loew LM. “Palette of fluorinated voltage-sensitive hemicyanine dyes.”
- Biochim Biophys Acta - Mol Cell Res. 2014; 1843:2284–2306. Oheim M, van ’t Hoff M, Feltz A, Za-maleeva A, Mallet JM, Collot M. “New red-fluorescent calcium indicators for optogenetics, photoactivation and multi-colour imaging.”
- Opt Express. 2009; 17:13354. Kobat D, Durst ME, Nishimura N, Wong AW, Schaffer CB, Xu C. “Deep tissue multiphoton microscopy using longer wavelength excita- tion.”
- J Mol Cell Cardiol. 2009; 46:811–820. Hom J, Sheu SS. “Morphological dynamics of mitochondria - A special emphasis on cardiac muscle cells.”
- Elife. 2016; 5:1–24. Dana H, Mohar B, Sun Y, Narayan S, Gordus A, Hasseman JP, Tsegaye G, Holt GT, Hu A, Walpita D, Patel R, Macklin JJ, Bargmann CI, Ahrens MB, Schreiter ER, Jayaraman V, Looger LL, Svoboda K, Kim DS. “Sensitive red protein calcium indicators for imaging neural activity.”
- Cardiovasc Res. 2017; 724–736. Kelly A, Salerno S, Connolly A, Bishop M, Charpentier F, Stolen T, Smith GL. “Normal interventricular differences in tissue architecture underlie right ventricular susceptibility to conduction abnormalities in a mouse model of Brugada syndrome.”
- Biomed Opt Express. 2016; 7:324. Perillo EP, McCracken JE, Fernee DC, Goldak JR, Medina FA, Miller DR, Yeh H-C, Dunn AK. Deep in vivo two-photon microscopy with a low cost custom built mode-locked 1060nm fiber laser.
or view regional numbers
QUOTE TOOL
enter stock numbers to begin
Copyright 2023 | Edmund Optics, Ltd Unit 1, Opus Avenue, Nether Poppleton, York, YO26 6BL, UK
California Consumer Privacy Acts (CCPA): Do Not Sell or Share My Personal Information
California Transparency in Supply Chains Act