Basics of Ultrafast Lasers
Authors: Olivia Wheeler
Table of Contents
- Unique Features of Ultrafast Lasers
- Technical Challenges of Ultrafast Lasers
- How Do You Know if You Need Pulse Compression?
- Pulse Compression Methods
- Ultrafast Laser Applications
- Attosecond Pulses: The Future of Ultrafast Lasers
Imagine trying to take a photo of a hummingbird with your cellphone. Just as you press the capture button, the hummingbird darts away leaving only a blur on your screen. Your phone camera simply is not fast enough to capture a crisp image of the hummingbird in flight.
Similar frustrations and limitations have motivated the scientific community to develop ultrafast lasers with short pulse durations on the order of femtoseconds to picoseconds (10-15 - 10-12 seconds). Instead of capturing a flying hummingbird, these lasers are used to capture much faster events like molecular vibrations, electronic motion, and even quantum phenomena.1,2,3 At timescales of one-millionth of a billionth of a second, ultrafast lasers expand our access to fundamental physical phenomena and revolutionize industrial processes. Understanding the unique features of ultrafast lasers, the key challenges facing these kinds of systems, and the available solutions for addressing those challenges enable system designers to choose the right ultrafast optics and create successful ultrafast optics.
Unique Features of Ultrafast Lasers
The ultrashort pulse durations of ultrafast lasers give these systems unique properties that distinguish them from longer pulse or continuous wave (CW) lasers. To generate such short pulses, it is necessary to have a broad spectral bandwidth. The pulse shape and central wavelength define the minimum bandwidth required to generate a pulse of a particular duration. In general, this relationship is described by the time-bandwidth product (TBP), which stems from the uncertainty principle. The TBP of a pulse with a Gaussian distribution is given by:
Δτ is the temporal duration of the pulse and Δν is the frequency bandwidth.4 Fundamentally, this equation states that there is a reciprocal relationship between spectral bandwidth and pulse duration, meaning that as pulse duration decreases, the bandwidth required to generate that pulse increases. Figure 1 demonstrates the minimum bandwidth required to support several different pulse durations.
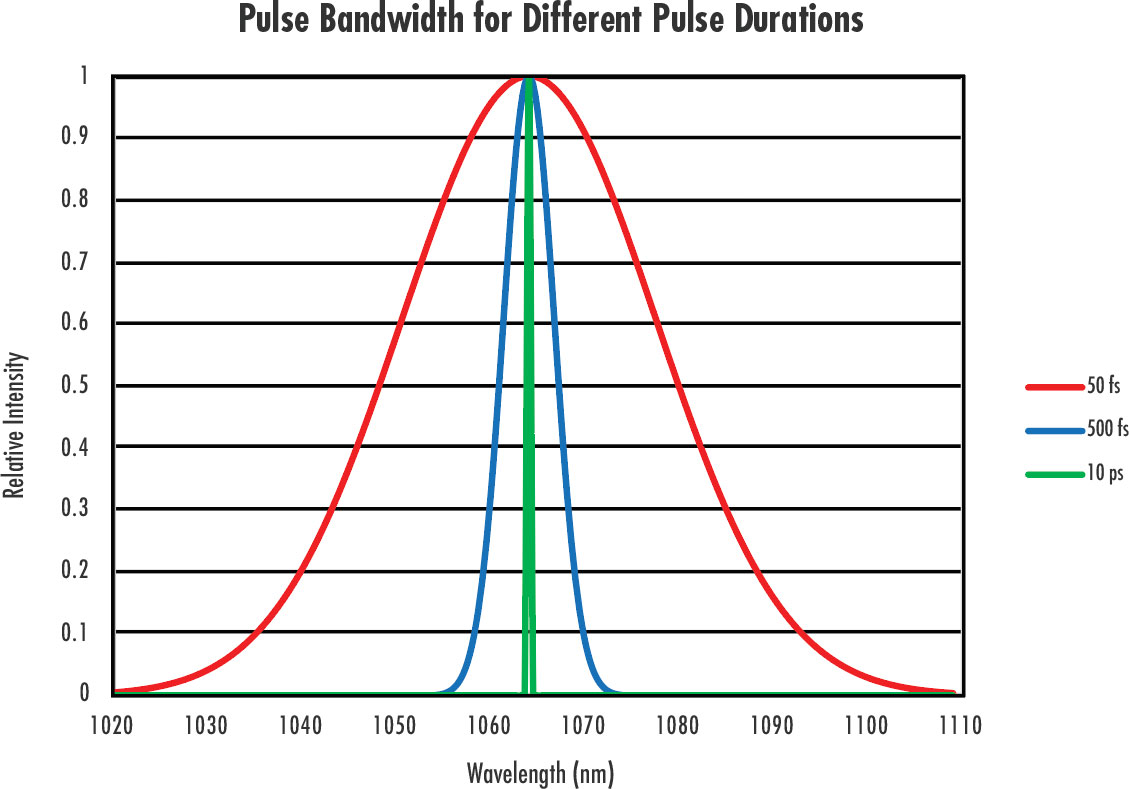
Figure 1: The minimum spectral bandwidths required to support 10ps (green), 500fs (blue), and 50fs (red) laser pulses.
In addition to broad spectral bandwidths, another feature of ultrashort pulse durations that is their incredibly high peak powers, which, although highly beneficial in many applications, may introduce some unique challenges. For context, let us examine the difference in the peak power output of a 10W CW laser vs. that of a 10W ultrafast laser with a 150fs pulse duration and repetition rate of 80MHz. The properties of this theoretical ultrafast laser are common for many commercially-available ultrafast lasers. For the CW laser, the average power and peak power are identical; the laser is always emitting 10W, or 10J/s. For the ultrafast laser, the average power is still 10W, just like that of the CW laser. However, the ultrafast laser is emitting that 10W of average power over only a small period. Figure 2 demonstrates the significant difference between average and peak power.
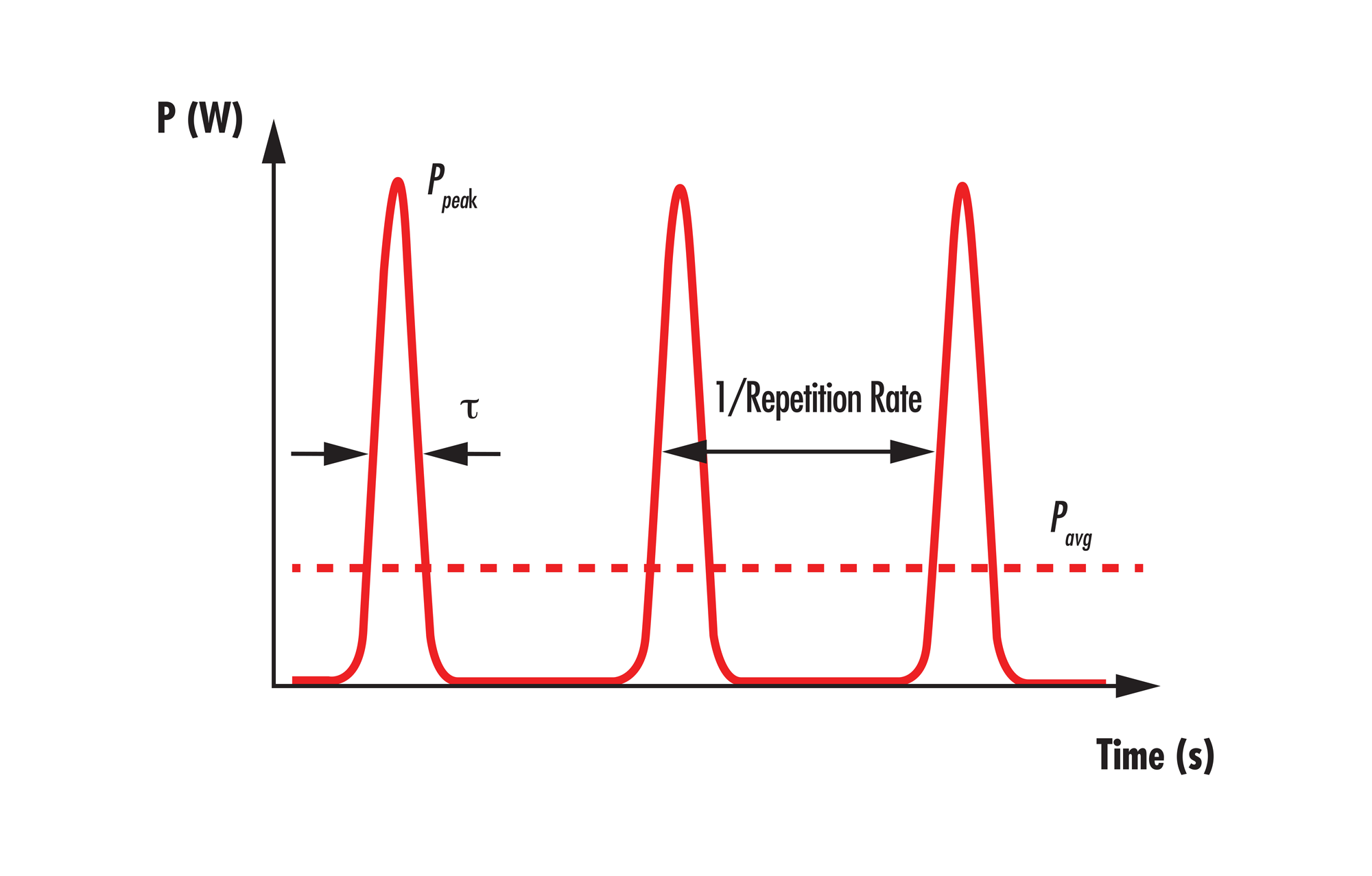
Figure 2: Depiction of average power, Pavg, and peak power, Ppeak, for a laser with pulse duration, t.
For the ultrafast laser, the 10W average power is distributed across the 80 million pulses emitted each second according to the repetition rate. At first glance, the sub-microjoule pulse energy of this laser may seem small and insignificant. However, when taking into account that this energy is condensed into only 150fs temporally, a massive peak power of over 800,000W is achieved. This is more than four orders of magnitude greater than the average power! While these immensely high peak powers and short pulse durations are the very features that have allowed ultrafast lasers to revolutionize a wide variety of applications, these traits also give rise to some of the unique technical challenges of working with ultrafast lasers.
Technical Challenges of Ultrafast Lasers
The broad spectral bandwidths, high peak powers, and short pulse durations of ultrafast lasers must be properly managed in your system. Typically, the simplest one of these challenges to address is the broad spectral output of the laser. If your work has mostly been with longer pulse or CW lasers in the past, your existing inventory of optical components may not reflect or transmit the full bandwidth of an ultrafast pulse. Edmund Optics® has a wide variety of ultrafast optics tailored specifically for these demanding ultrafast systems.
Laser Damage Threshold (LDT)
The laser damage threshold (LDT) of ultrafast optics is also significantly different and more difficult to navigate than that of more conventional laser sources (Figure 3). When sourcing optics for nanosecond pulse lasers, LDT values are commonly on the order of 5-10 J/cm2. For ultrafast optics, values this large are practically unheard of, as LDT values will more likely be on the order of < 1J/cm2, typically closer to 0.3J/cm2. This dramatic variation in LDT magnitude for different pulse durations is a result of the pulse duration-based laser damage mechanisms at play. For nanosecond lasers or those with even longer pulses, the main mechanism that leads to damage is thermal heating. The optic’s coating and substrate material absorb incident photons and heat up. This can result in a deformation of the material’s lattice. Effects such as thermal expansion, cracking, melting, and lattice strain are common thermal damage mechanisms for these types of laser sources.5
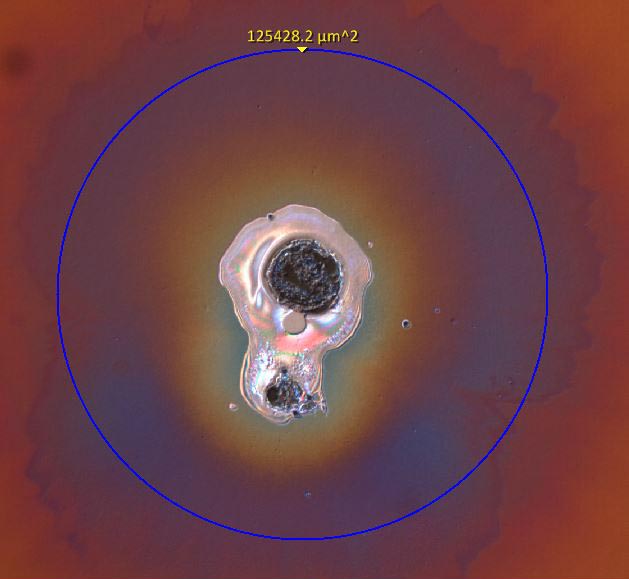
Figure 3: Laser-induced damage to optical surfaces, like the damage shown here, can decrease the performance of laser systems, render them useless, and even be dangerous. The damage mechanisms when using ultrafast lasers differ significantly from those of longer pulse lasers because of the short pulse durations.
However, for ultrafast lasers, the pulse duration itself is faster than the timescale of heat transfer from the laser to the material lattice, so thermal effects are not the primary cause of laser-induced damage (Figure 4). Instead, the high peak powers of ultrafast lasers shift the damage mechanism to nonlinear processes like multiphoton absorption and ionization.6 This is also why one cannot simply scale the LDT rating for nanosecond pulses down to that for ultrafast pulses, as the physical mechanisms of damage are different. Because of this, the best optic for your specific application would be one with a sufficiently high LDT rating that was obtained under the same use conditions like wavelength, pulse duration, and repetition rate. Optics tested under different conditions will not be representative of the real performance of the same optics in your system.
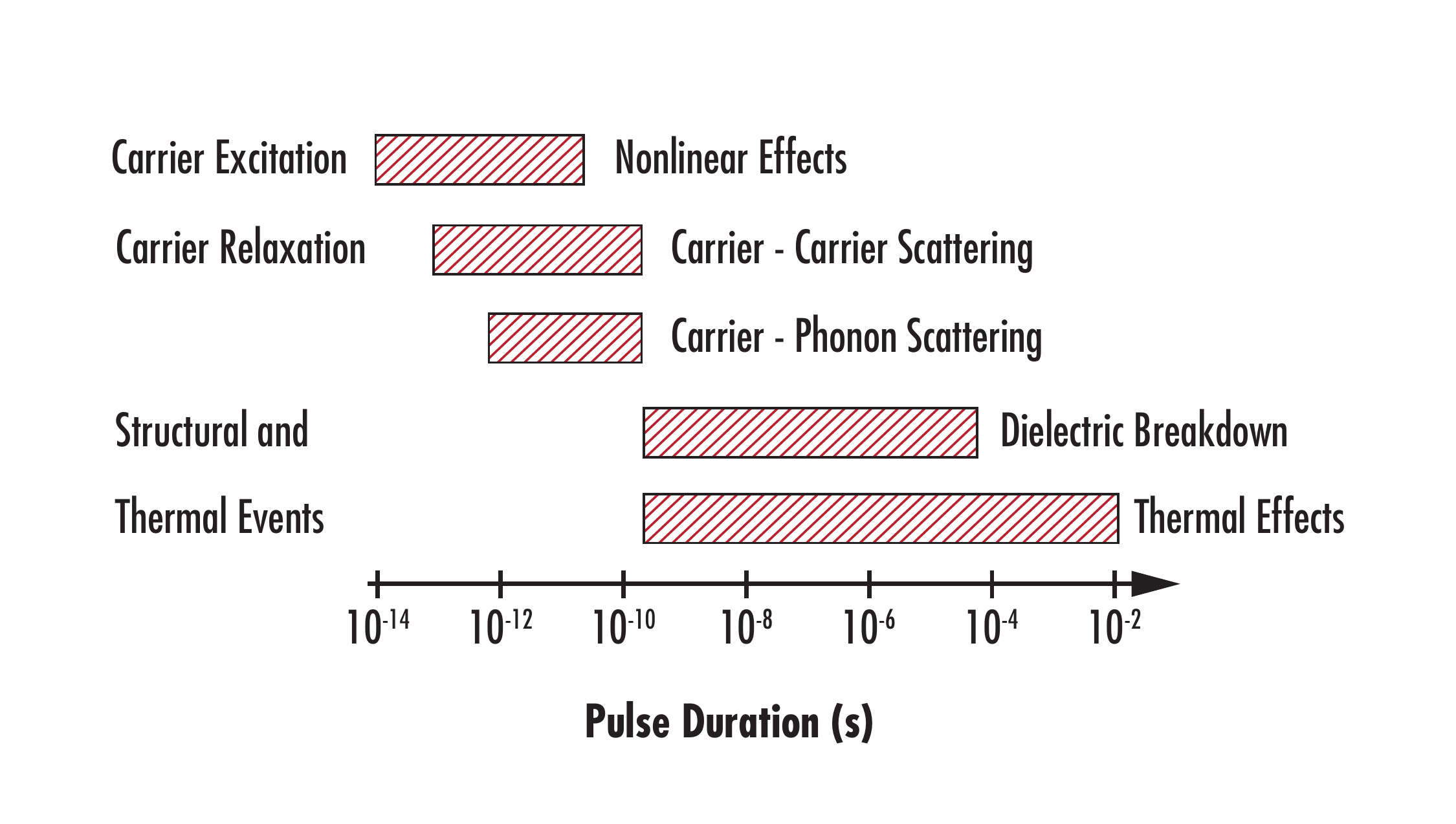
Figure 4: Mechanisms for laser-induced damage across different pulse durations.
Dispersion and Pulse Spreading: Group Delay Dispersion
One of the most difficult technical challenges encountered when using ultrafast lasers is maintaining the ultrashort pulse durations initially emitted by the laser. Ultrafast pulses are highly susceptible to temporal distortion which elongates the pulses. This effect gets worse as the initial pulse duration gets shorter. While an ultrafast laser may emit pulses with a 50fs duration, relaying this pulse to a target position using mirrors and lenses, or even just transmitting the pulse through air, has the potential to broaden the pulse temporally.
This temporal distortion is quantified using a metric called group delay dispersion (GDD), which is also known as second-order dispersion. In reality, there are higher-order dispersion terms that may affect the temporal profile of the ultrafast laser pulse too, but in practice it is often sufficient to examine the impact of GDD. GDD is a frequency-dependent value that, for a given material, scales linearly with thickness. Transmissive optics like lenses, windows, and objective assemblies typically have positive GDD values, which indicates that the once-compressed pulse may leave the transmissive optical component with a longer pulse duration than was emitted from the laser system. Lower frequency (i.e., longer wavelength) components travel faster than the higher frequency (i.e., shorter wavelength) components of the pulse. As the pulse travels through more and more material, the wavelengths in the pulse will continue to stretch further and further apart in time. For shorter pulse durations, and therefore broader bandwidths, this effect is further exaggerated and can lead to significant temporal distortion of the pulse.
For longer pulses with nanosecond and even picosecond pulse durations, GDD is not a major concern. However, for shorter femtosecond pulses, even a 10mm thick piece of N-BK7 in the beam path can broaden a 50fs pulse centered at 800nm by over 12%! This is roughly equivalent to having two windows or filters in the beam path
The impact of GDD on your application depends on several factors, including input pulse duration (τinput), central frequency (or wavelength), and the material in which the pulse is propagating. Temporal stretching due to GDD is described by
Equation 1 makes it clear that shorter pulse durations will be more significantly broadened compared to longer input pulse durations for the same GDD value. This is why GDD is not discussed in the context of nanosecond or picosecond pulses. For example, it would take 20,000fs2 of GDD to broaden a 1ps pulse by only 0.2%. The example in the following paragraph shows that this would be equivalent to propagating a 1030nm pulse through more than 1m of fused silica.
The refractive index of a material depends on the frequency of light passing through it, and GDD has a similar dependence on the refractive index. When selecting both transmissive and refractive optics for use in an ultrafast system, it is often recommended to use fused silica, as this has one of the lowest GDD values in the visible and NIR wavelength ranges. For example, propagating a 1030nm pulse through 1mm of fused silica will generate ~19fs2 of GDD, but 1mm of SF11 will lead to a GDD of more than 125fs2 at the same wavelength.7,8 Refractive index databases, such as refractiveindex.info, are helpful resources for determining which material is the best choice for your optics, as well as how much GDD you are accumulating in your beam path.
Because of this tendency towards positive GDD and temporal distortion, it is highly recommended to use specialized ultrafast optics which impart minimal to no additional GDD and therefore decrease the chances of an elongated pulse duration.
How Do You Know if You Need Pulse Compression?
When is it necessary to (re)compress your laser pulse? Blurry images in ultrafast imaging applications like multiphoton microscopy indicate that pulses may be stretching temporally. In ultrafast laser machining, pulse stretching can lead to less accurate cuts and less precision. Elongating pulse duration lowers the probability of multiphoton interactions, thereby reducing the efficiency of an ultrafast system. While it is impossible to provide hard and fast rules for every situation, the example calculation below helps demonstrate some best practices for determining if pulse compression is needed.
Consider a multiphoton microscopy setup with the beam path shown in Figure 5.
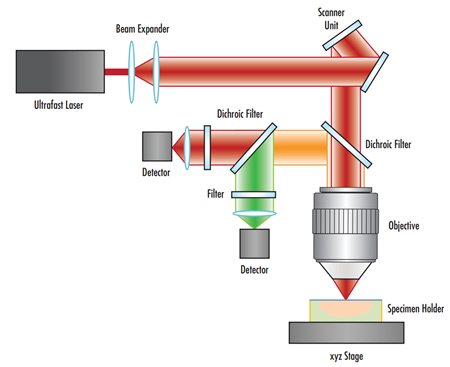
Figure 5: Example schematic of the beam path in a multiphoton microscopy experiment.
A first-order approximation of the amount of pulse elongation may be obtained by summing the GDD contribution of all elements in the system before the laser reaches the specimen. Let us assume that the major contributors to dispersion will be the beam expander, dichroic filter, and focusing objective. We will disregard the impact of the scanner mirrors, as they are typically made from low GDD metallic coatings. If the pulse is centered at a wavelength of 1030nm, this system could easily add more than 600fs2 of GDD.
Whether or not the pulses in your system need to be compressed depends on the input pulse duration and the specific needs of your application. If starting with a 150fs pulse, transmitting through the optics would have a negligible effect on pulse duration. However, if your application requires time resolution that is only accessible with a 10fs laser pulse, this amount of GDD would stretch your initial pulse to about 167fs. In that case, recompression is needed. These exact details heavily depend on your specific beam path and application. For guidance in choosing the best optical components for your pulse duration and application, please contact us.
Pulse Compression Methods
In the case where you need to (re)compress your ultrafast laser pulses, there are several different approaches. The sections below contain a summary, including advantages and disadvantages, of several common techniques for pulse (re)compression.
Prism and Grating Compressors
Prisms compress ultrafast pulse durations by applying a frequency-dependent delay to the various frequency components of the pulse because of dispersion, or the difference in refractive index experienced by different wavelengths (Figure 6). The resulting optical path difference aligns the different wavelengths of the pulse in time. Grating compressors compress pulses using a similar mechanism, but they rely on diffraction rather than refraction to treat wavelengths differently and recompress the pulse. However, both prism and grating compressors can be difficult to align and they can apply higher order dispersion to the pulses, further distorting their temporal profiles.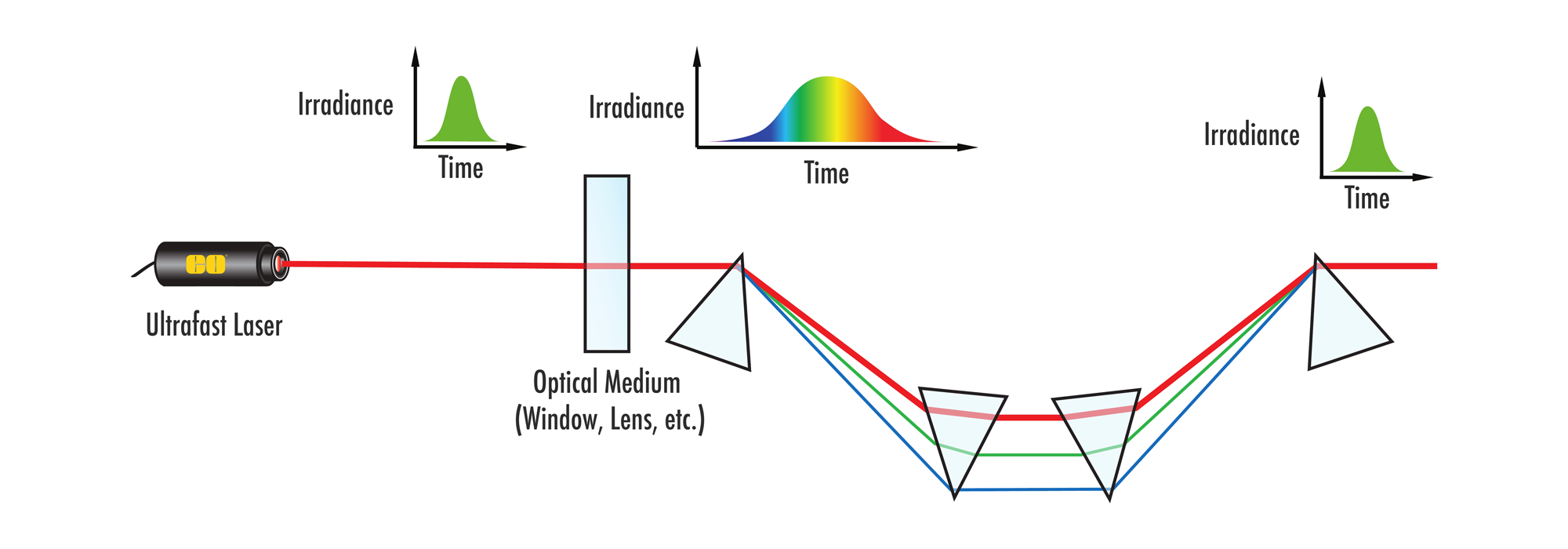
Figure 6: While prisms and gratings can be used for pulse compression, they face a number of disadvantages compared to highly-dispersive mirrors, which will be discussed later.
Advantages
- Continuously tunable via variables like distance between elements, amount of material of prism used, and grating groove density
- Can accommodate very large bandwidths
- Good for adjusting very, very short pulses
Disadvantages
- Difficult to align for beginners
- Apply higher-order dispersion, which can further distort a pulse’s temporal profile
- Can easily create separate frequency components of the pulse in space (i.e., generate “spatial chirp”)
Pulse Compressing Mirrors: Chirped Mirrors
Chirped mirrors are one of two types of pulse-compressing mirrors that we will discuss. They function by creating a wavelength-dependent penetration depth into the coating of the mirror (Figure 7). Similar to the principle behind prism and grating compressors, chirped mirrors delay some wavelengths differently relative to others. This effect arises because some wavelengths travel deeper into the coating. The initial positive dispersion is corrected for and all wavelengths end up emerging from the coating at the same time. At this point, the pulse is recompressed. Chirped mirrors are typically characterized by high GDD oscillations, so they should be used in pairs of complementary chirped mirrors to get a semi-flat GDD output.
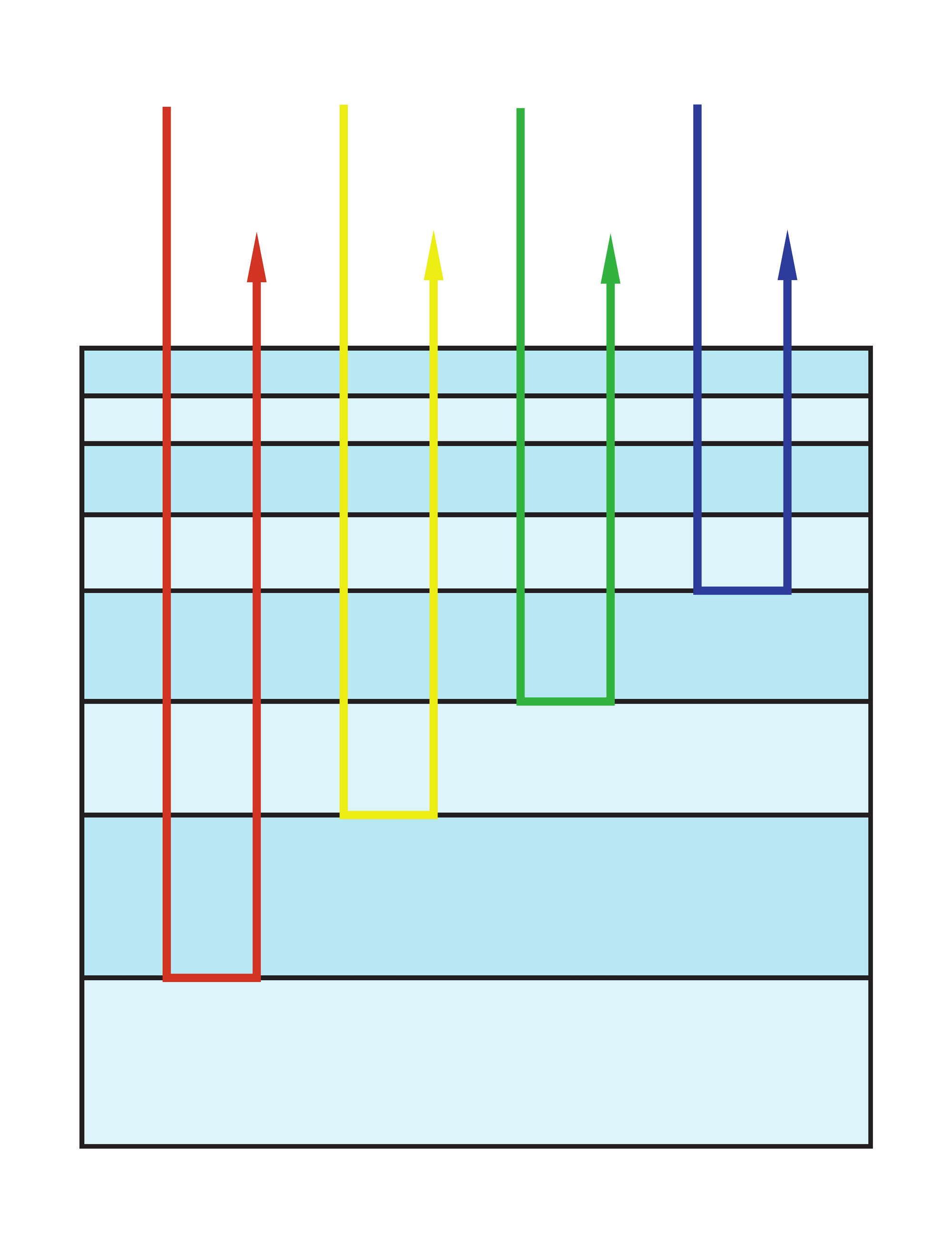
Figure 7: The variable layer thickness of chirped mirrors, combined with strategic choice of coating materials, applies a negative dispersion to an incoming pulse.
Advantages
- Broad bandwidths possible
- Typically have small angles of incidence, making it easy to reflect many times between the two mirrors in the chirped mirror pair
- Easy to align relative to prisms and gratings
Disadvantages
- Provide only integral steps of GDD and are not continuously tunable
- Have to use in a complementary pair due to GDD oscillations
- Limited in application by their specified bandwidth
- Typically feature smaller magnitudes of GDD than other methods
For example, a chirped mirror pair would be a good choice when compensating for a small amount of GDD or switching between different setups, like between a Ti:Sapphire and Yb-doped laser. Figure 8 shows a sample GDD plot of a complementary chirped mirror pair, where “L” and “S” indicate the responses of the individual elements of the pair and “∑” indicates their combined response.
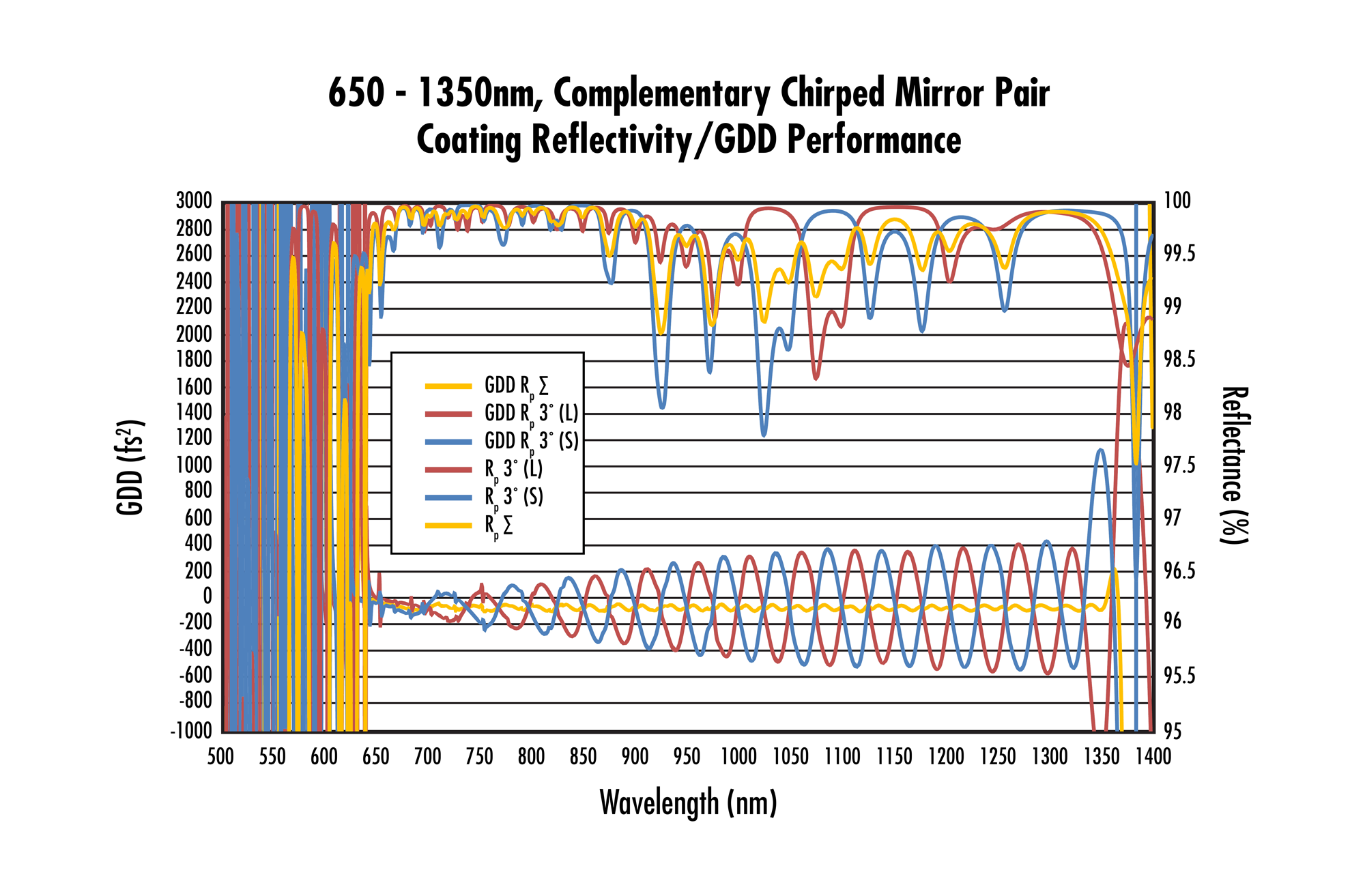
Figure 8: The high-magnitude GDD oscillations in these ultra-broadband chirped mirrors are offset by using the “L” and “S” components in concert to achieve the combined response, “∑”.
Pulse Compressing Mirrors: Highly-Dispersive Mirrors
Highly-dispersive mirrors combine the frequency-dependent penetration depth of chirped mirrors with a multi-resonance effect to produce larger magnitudes of GDD with less oscillations. While the bandwidths addressed by highly-dispersive mirrors may be somewhat narrower than the methods discussed above, many highly-dispersive mirror designs can achieve extremely high reflectivities across their specified bandwidth (Figure 9).
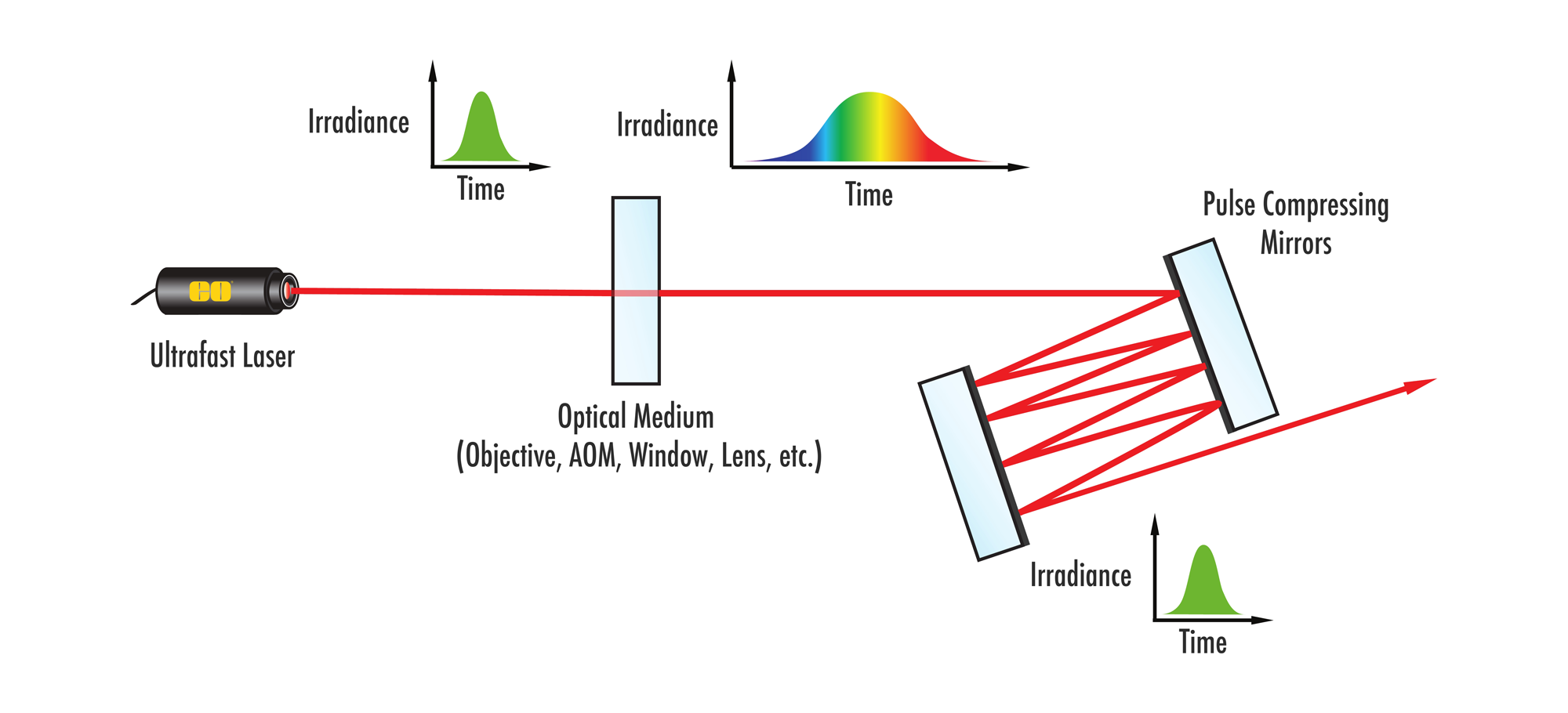
Figure 9: Highly-dispersive mirrors are powerful tools for introducing negative dispersion, canceling out the positive dispersion experienced by ultrafast laser pulses as they transmit through optical media.
Advantages
- Able to achieve high magnitudes of GDD
- Typically feature small angles of incidence for many reflections between multiple mirrors
- Easy to align compared to prisms and gratings
- Do not need to use in pairs like complementary chirped mirrors
- Typically feature high reflectivity and therefore generate less light loss throughout the system
Disadvantages
- Provide only integral steps of GDD that are not continuously tunable
- Limited by their specified bandwidth
Highly-dispersive mirrors are ideal options for compensating for a high amount of GDD in a fixed system. Sample reflectivity and GDD curves for a highly-dispersive mirror are shown in Figures 10 and 11.
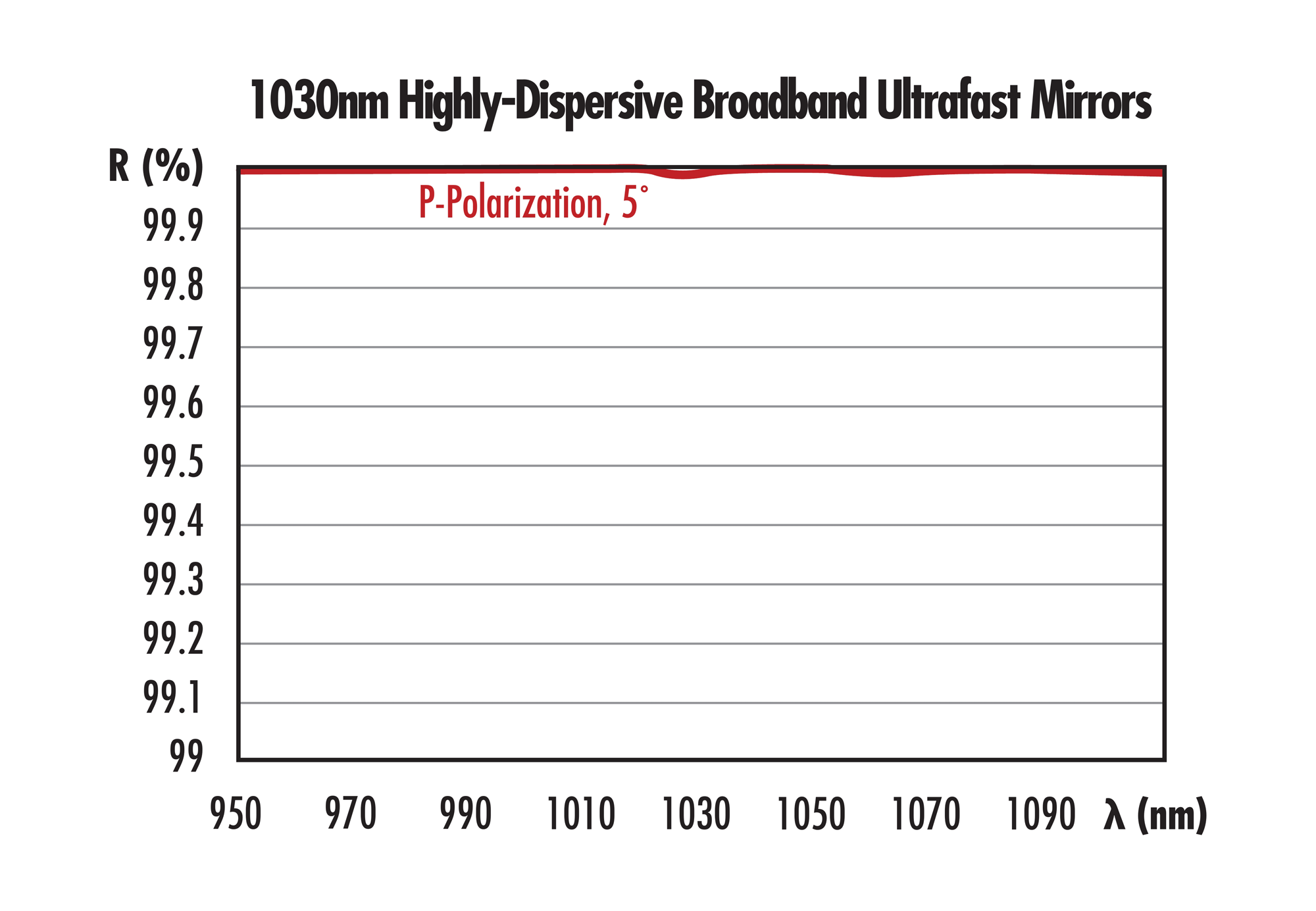
Figure 10: In addition to negative dispersion for ultrafast pulse compression, highly-dispersive mirrors provide high reflectivities to maximize throughput.
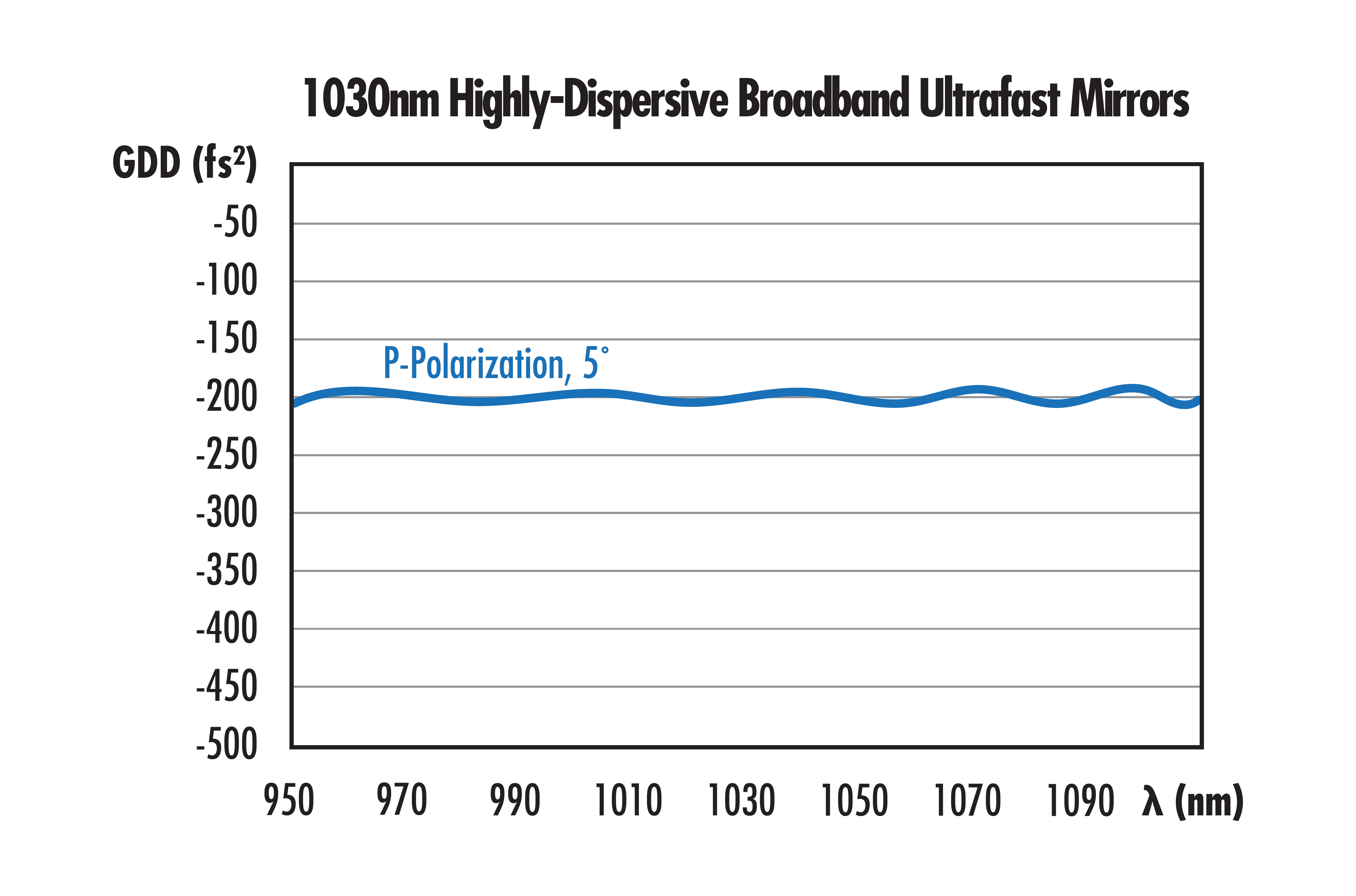
Figure 11: Ultrafast highly-dispersive mirrors offer negative GDD with a high magnitude that has far less wavelength-dependent oscillations compared to chirped mirrors
The decision of when and how to recompress ultrafast laser pulses is heavily application-dependent, but a basic knowledge of different methods and their advantages/disadvantages gives you a head start. Again, contact us for guidance on determining the best pulse compressing method for your system.
Ultrafast Laser Applications
Spectroscopy
Since the introduction of ultrafast laser sources, spectroscopy has been one of their primary applications. By shrinking the pulse duration down to femtoseconds and even attoseconds, dynamic processes in physics, chemistry, and biology that were historically impossible to observe are now accessible. One of these key processes is atomic motion, and observing this has improved scientific understanding of fundamental processes such as molecular vibrations, molecular dissociation, and energy transfer in photosynthetic proteins.9,10
Biological Imaging
Ultrafast lasers’ high peak powers support nonlinear processes that improve resolution in biological imaging, such as in multiphoton microscopy (Figure 12). In multiphoton systems, two photons must be overlapped in both space and time for the generation of a nonlinear signal from the biological medium or fluorescent target. This nonlinear mechanism increases imaging resolution by substantially reducing the background fluorescence signal that plagues studies conducted with single photon processes.11 Figure 13 illustrates this reduced signal background. The smaller excitation area of multiphoton microscopy also prevents phototoxicity and minimizes damage to the sample.
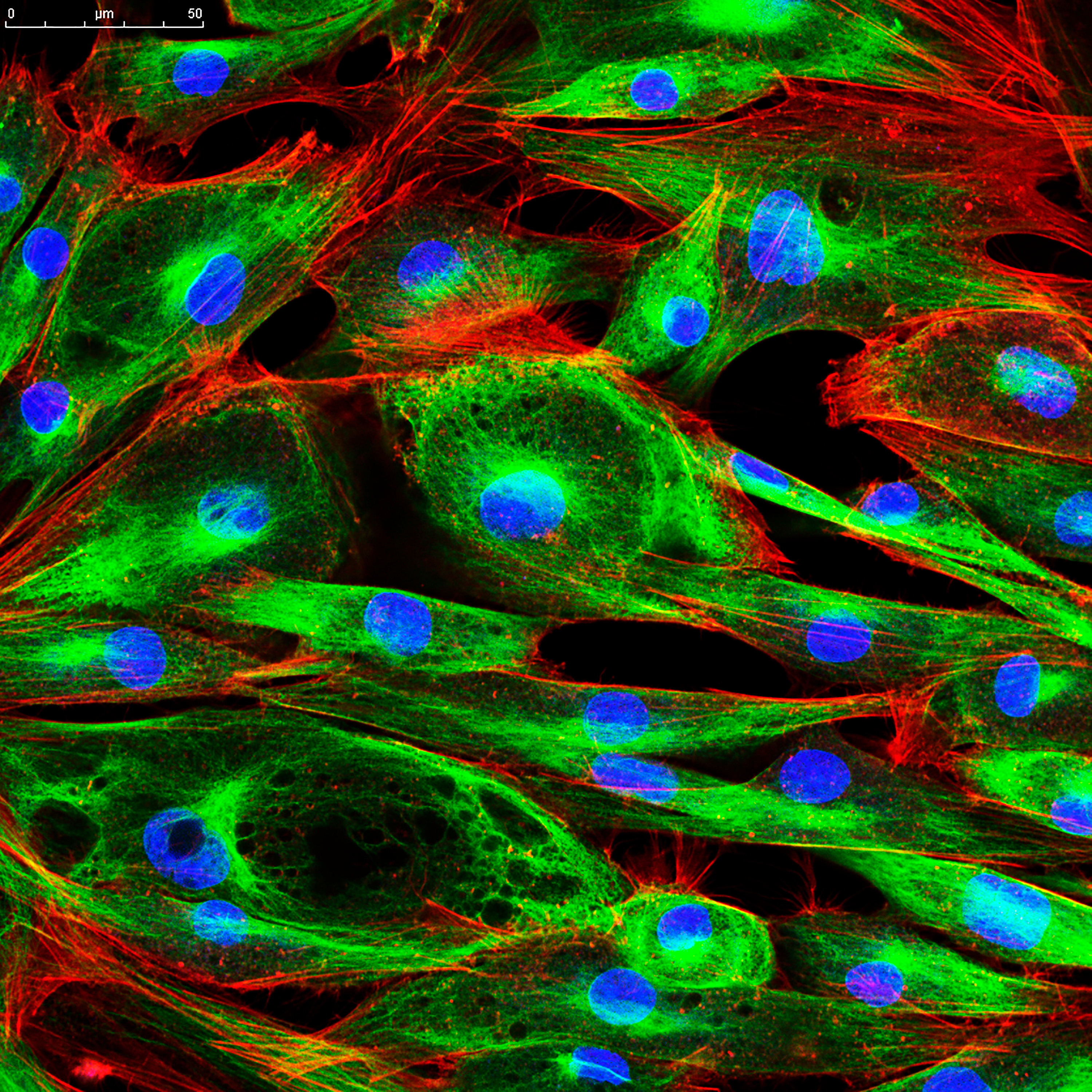
Figure 12: Multiphoton, or non-linear, microscopy uses ultrafast laser sources to capture high-resolution three-dimensional (3D) images with reduced photobleaching and phototoxicity compared to traditional confocal microscopy techniques.
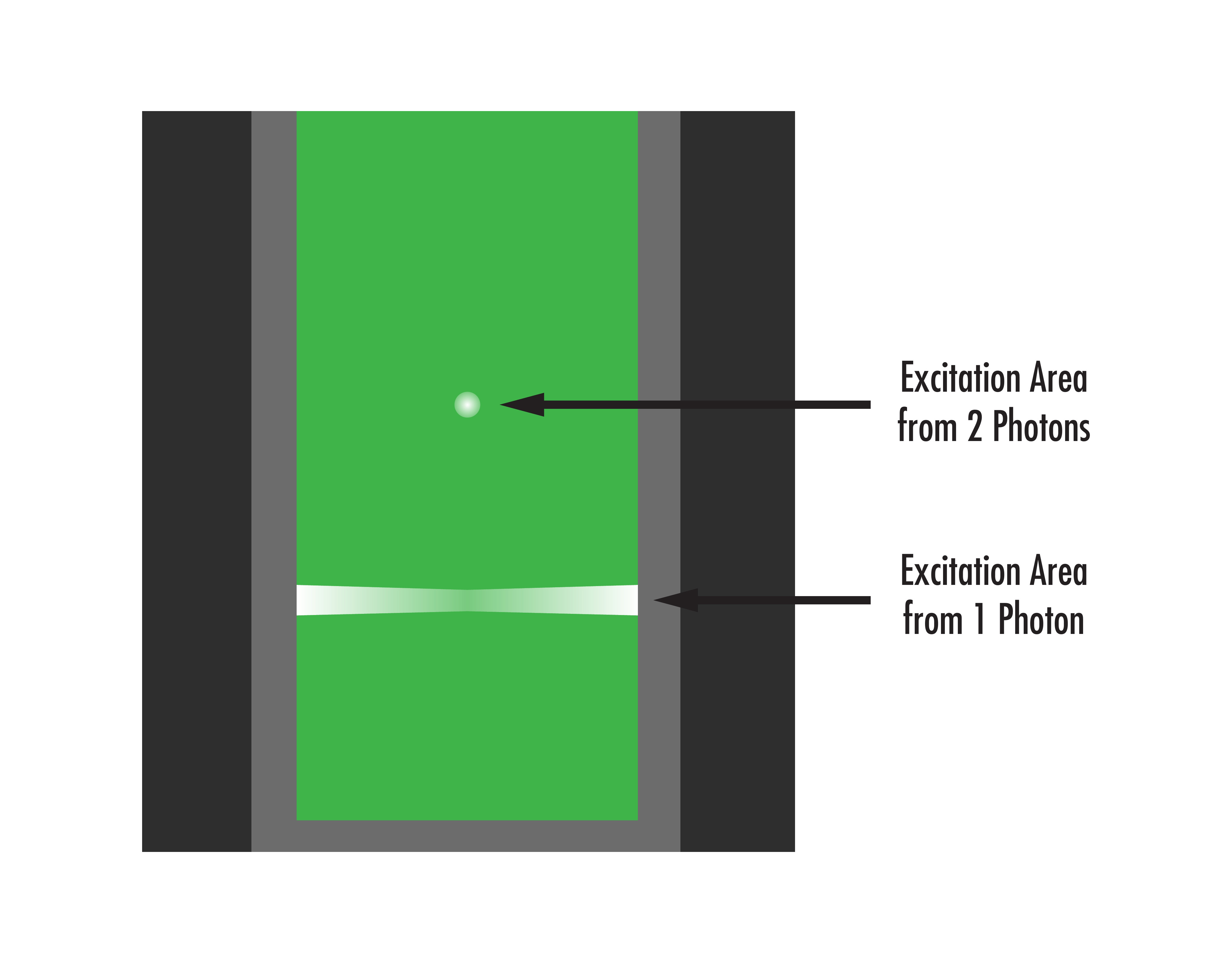
Figure 13: Depiction of the signal location for two-photon two-photon (top) and single-photon (bottom) microscopy systems. The overlap produced by two photons results in a smaller excitation volume, while the single-photon signal suffers from background signal from outside the focal plane.
Laser Materials Processing
Ultrafast laser sources have also revolutionized laser micromachining and materials processing because of the unique ways in which ultrashort pulses interact with materials. As mentioned previously when discussing LDT, ultrafast pulse durations are faster than the time scale of thermal diffusion into a material lattice. Compared to nanosecond pulse lasers, ultrafast lasers produce a much smaller heat-affected zone, resulting in lower kerf loss and more precise machining.12 This principle also applies to medical applications, where the increased precision of ultrafast laser cuts helps decrease damage to surrounding tissues and improves the patient experience in laser surgery.13
Attosecond Pulses: The Future of Ultrafast Lasers
As research continues into advancing ultrafast lasers, new and improved sources with even shorter pulse durations are being developed. In order to gain insights into even faster physical processes, many researchers are focusing on the generation of attosecond pulses—pulses on the order of 10-18 seconds in the extreme ultraviolet (XUV) wavelength region. Attosecond pulses allow for the tracking of electronic motion and improve our understanding of electronic structure and quantum mechanics.14 While the integration of XUV attosecond lasers into industrial processes has not yet gained significant traction, ongoing research and advancements in this field will almost certainly propel this technology out of the lab and onto the manufacturing floor, as has been the case with femtosecond and picosecond laser sources.
Off-the-Shelf Ultrafast Optics

- Pulse compressing optics (highly dispersive mirrors, chirped mirrors, gratings, and prisms)
- Lenses, filters, polarizers, and more
- Reflective objectives and beam expanders
Custom Ultrafast Optics Manufacturing
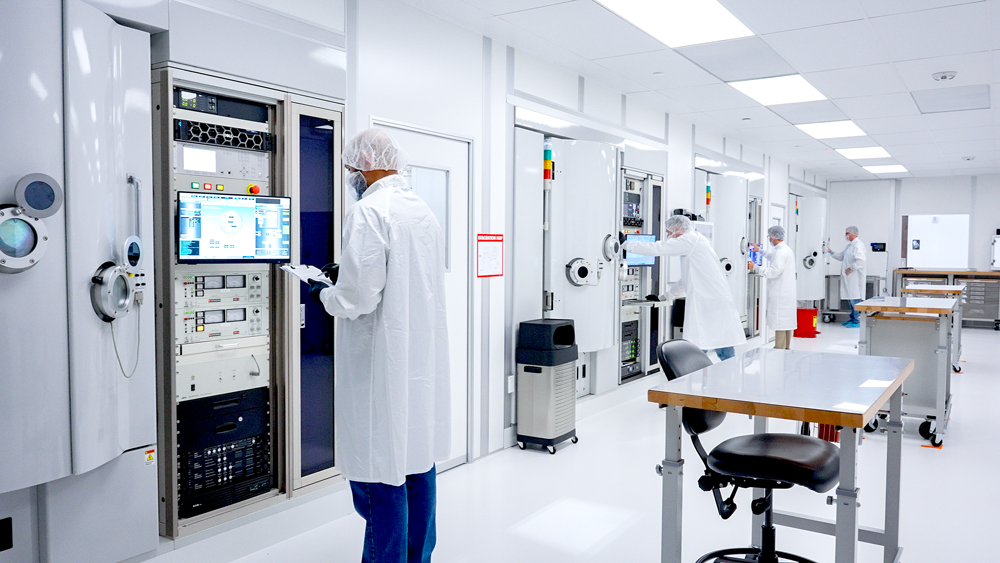
- Highly-dispersive mirrors, low GDD optics, and beam expanders
- Intra- and extra-cavity optics for high-power ultrafast lasers
- Third order dispersion of 0 fs3, or negative values down to -2500 fs3
- Cost-effective ultrafast-enhanced silver coatings with R>99% and GDD as low as 0 ±20fs2 over common ultrafast wavelengths
References
- Bredenbeck et al. “Labeling Vibrations by Light: Ultrafast Transient 2D-IR Spectrosocpy Tracks Vibrational Modes during Photoinduced Charge Transfer.” J. Am. Chem. Soc. 2004, 126, 5, 990-991
- Camargo et al. “Visualizing Ultrafast Electron Transfer Processes in Semiconductor-Metal Hybrid Nanoparticles: Toward Excitonic-Plasmonic Light Harvesting.” Nano Lett. 2021, 21, 3, 1461-1468.
- Maiuri et al. “Ultrafast Spectroscopy: State of the Art and Open Challenges.” J. Am. Chem. Soc. 2020, 142, 3-15.
- Lazaridis et al. “Time-bandwidth product of chirped sech2 pulses: application to phase-amplitude-coupling factor measurement.” Opt. Lett. 1995, 20, 10, 1160-1162.
- Wood, R. “Laser-Induced Damage by Thermal Effects.” Laser-Induced Damage in Optical Materials. 2014, 9-24.
- Jing et al. “Calculation of femtosecond pulse laser induced damage threshold for broadband antireflective microstructure arrays.” Optics Express. 2009, 17, 26, 24137-24152.
- I. H. Malitson. Interspecimen comparison of the refractive index of fused silica, J. Opt. Soc. Am. 55, 1205-1208 (1965).
- SCHOTT Zemax catalog 2017-01-20b (obtained from http://www.schott.com)
- Geßner et al. “Femtosecond Multidimensional Imaging of a Molecular Dissociation.” Science Reports. 2006, 311, 219-222.
- Zigmantas et al. “Ultrafast laser spectroscopy uncovers mechanisms of light energy conversion in photosynthesis and sustainable energy materials.” Chem. Phys. Rev. 2022, 3, 041303.
- Sheppard, C. J. R. “Multiphoton microscopy: a personal historical review, with some future predictions.” J. Biomed. Opt. 202, 1, 014511.
- Phillips et al. “Ultrafast laser processing of materials: a review.” Advances in Optics and Photonics. 2015, 7, 4, 684-712.
- Hoy et al. “Clinical Ultrafast Laser Surgery: Recent Advances and Future Directions.” IEEE Journal of Selected Topics in Quantum Electronics. 2014, 20, 7100814.
- Li et al. “Attosecond science based on high harmonic generation from gases and solids.” Nature Comm. 2020, 11, 2748.
More Resources
- Ultrafast Dispersion Application Note
- Highly-Dispersive Mirrors Application Note
- Ultrafast Laser Applications: Short Pulse Revolution Webinar Recording
- Choosing the Right Lasers and Optics for Ultrafast Microscopy Webinar Recording
- Ultrafast Optics: Challenges and Solutions Webinar Recording
- Ultrafast Lasers – The Basic Principles of Ultrafast Coherence Application Note
- LIDT for Ultrafast Lasers Application Note
or view regional numbers
QUOTE TOOL
enter stock numbers to begin
Copyright 2023 | Edmund Optics, Ltd Unit 1, Opus Avenue, Nether Poppleton, York, YO26 6BL, UK
California Consumer Privacy Acts (CCPA): Do Not Sell or Share My Personal Information
California Transparency in Supply Chains Act